"We are made of star stuff", as Carl Sagan once said. But what did he mean by that, and if it's true, where does 'star stuff' come from?
Another way of asking this question takes us right back to basics.
Everything we can see in the world around us is made of atoms. But where did those atoms come from?
This has fascinated natural philosophers and scientists for centuries, and it's a question we’re still learning more about today.
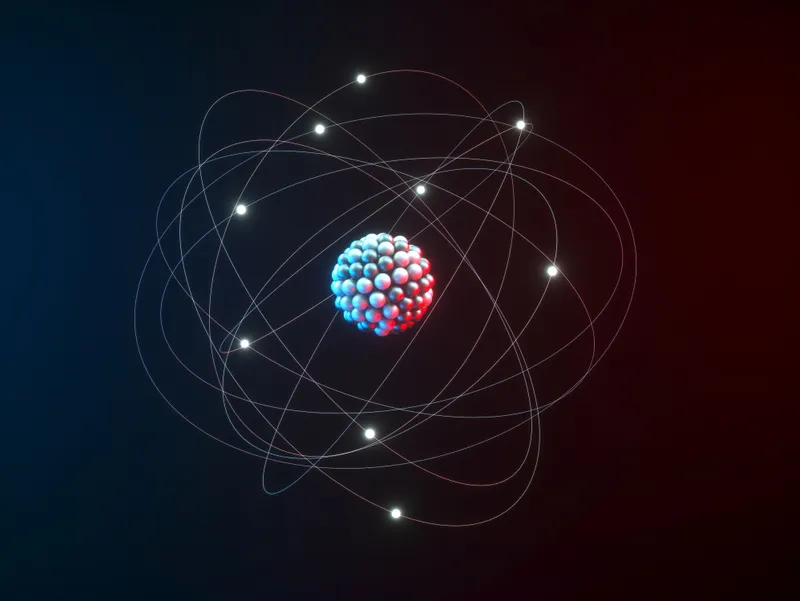
At the start of the 18th century, Isaac Newton declared that atoms had probably been created by God at the beginning of time.
He believed that atoms were indestructible: "no ordinary power being able to divide what God had made one in the first creation."
However, in the first decades of the 20th century, physicists found that they could break atoms apart in the lab using powerful, albeit earthly, forces, which eventually led to the realisation that every atom in the periodic table is made of just three subatomic particles: electrons, protons and neutrons.
The discovery of atomic substructure opened up the possibility that atoms themselves had been created out of these basic constituents. But how?
Every atom has the same structure, a tiny positively charged nucleus orbited by negatively charged electrons.
The nucleus itself is made of positively charged protons and electrically neutral neutrons bound together by the strong nuclear force, and crucially it is the number of protons in the nucleus that determines whether an atom is, say, carbon, oxygen or uranium.
Star stuff - back to basics
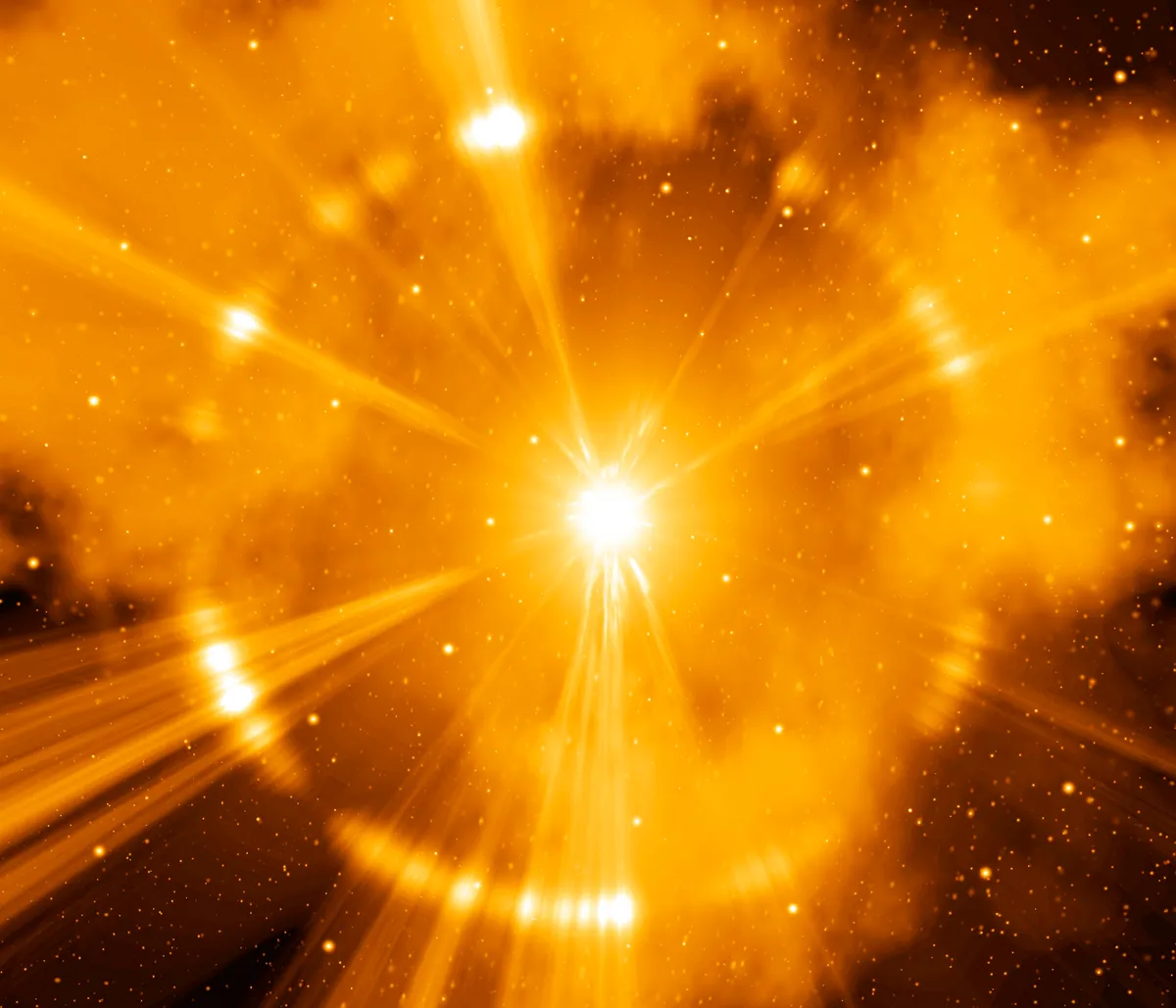
Hydrogen is the simplest atom, a single proton orbited by a single electron, and most of the hydrogen we see in the Universe today was created shortly after the Big Bang.
It is the obvious candidate for the raw material from which all the heavier elements are made.
The next simplest atom is helium, whose nucleus is made of two protons and two neutrons.
However, combining hydrogen together to make helium is far from easy.
Protons are positively charged, and so exert an enormous repulsive force on each other when they come close together.
For two protons to fuse, they have to get within around 10-15 metres of each other – a distance comparable to the size of the protons themselves – at which point the attractive strong nuclear force begins to overcome their electrical repulsion.
However, to get two protons this close they have to be moving incredibly quickly, fast enough to zip up the steeply rising electrical barrier that keeps them apart.
This means that for hydrogen to undergo nuclear fusion it has to be very, very hot indeed, with a temperature of at least several million degrees.
Where in the cosmos can such extreme conditions be found? One answer is, in the interiors of stars.
Stars, nuclear fusion and helium

Take, for example, the Sun. At its centre, the crushing gravity of our local star’s mass heats the core to a blistering 15 million degrees, allowing hydrogen nuclei (protons) to fuse together to produce helium.
However, since you need two protons and two neutrons to make helium, this doesn’t happen in a single reaction but in a step-by-step process known as the ‘proton–proton chain’, whereby a helium nucleus is built up through a series of nuclear collisions, accompanied by the occasional conversion of a proton into
a neutron.
Not only do these nuclear fusion reactions produce helium, they also generate the energy that keeps our local star shining and stops it collapsing under its own gravity.

The Sun will carry on merrily fusing hydrogen into helium for another five billion years, but eventually the hydrogen needed to fuel its nuclear furnace will run out.
At that point, things will get rather interesting for anyone still living in the inner Solar System.
Without a source of energy to counterbalance gravity, the Sun’s core will start to collapse and heat up.
Eventually, it will become so hot that hydrogen fusion will re-ignite, but this time in a spherical shell surrounding the helium-rich core.
Previously, this layer was too cool for fusion to take place.
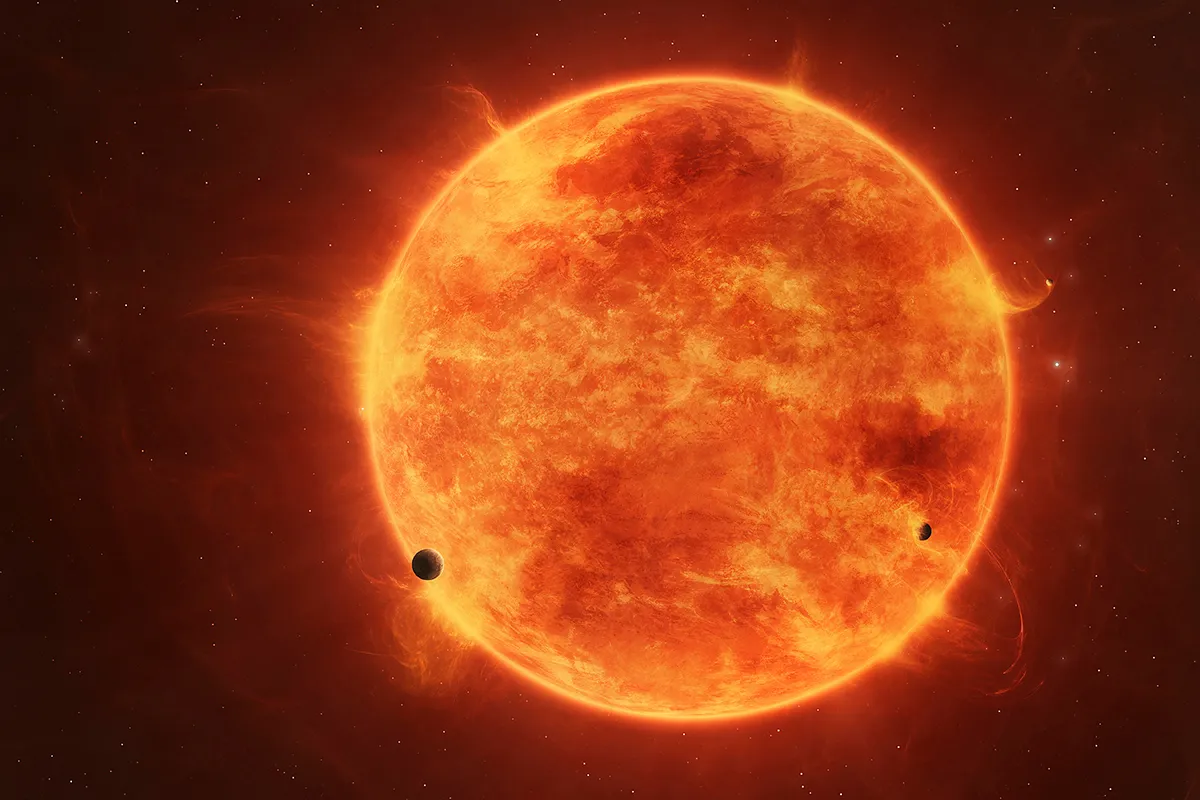
Death throes of a star
The restart of fusion will release a new blaze of light into the upper regions of the star, inflating it to become a monstrous red giant between 100 and 200 times its current diameter, so large that it will consume the inner planets, likely including Earth.
Meanwhile, deep inside the red giant, the core will continue to shrink and heat up, eventually reaching a searing 100 million degrees.
This is hot enough to allow even larger atoms to combine, igniting helium fusion into carbon, and then carbon–helium fusion to make oxygen.
When this happens, the Sun will shrink back down to a yellowish star, around 10 times its current size.
However, this new age of helium fusion will be brief in solar terms, a mere 100 million years, after which the core will resume its inward collapse.
In its final gasps, mixing between the star’s inner layers will result in the fusion of carbon and hydrogen to make nitrogen, until at last, the Sun will gently puff its atmosphere out into space, enriching the surrounding galactic medium in carbon and nitrogen.
At the centre of this expanding cloud of luminous gas will be all that remains of the solar core, a glowing husk about the size of Earth: a white dwarf.
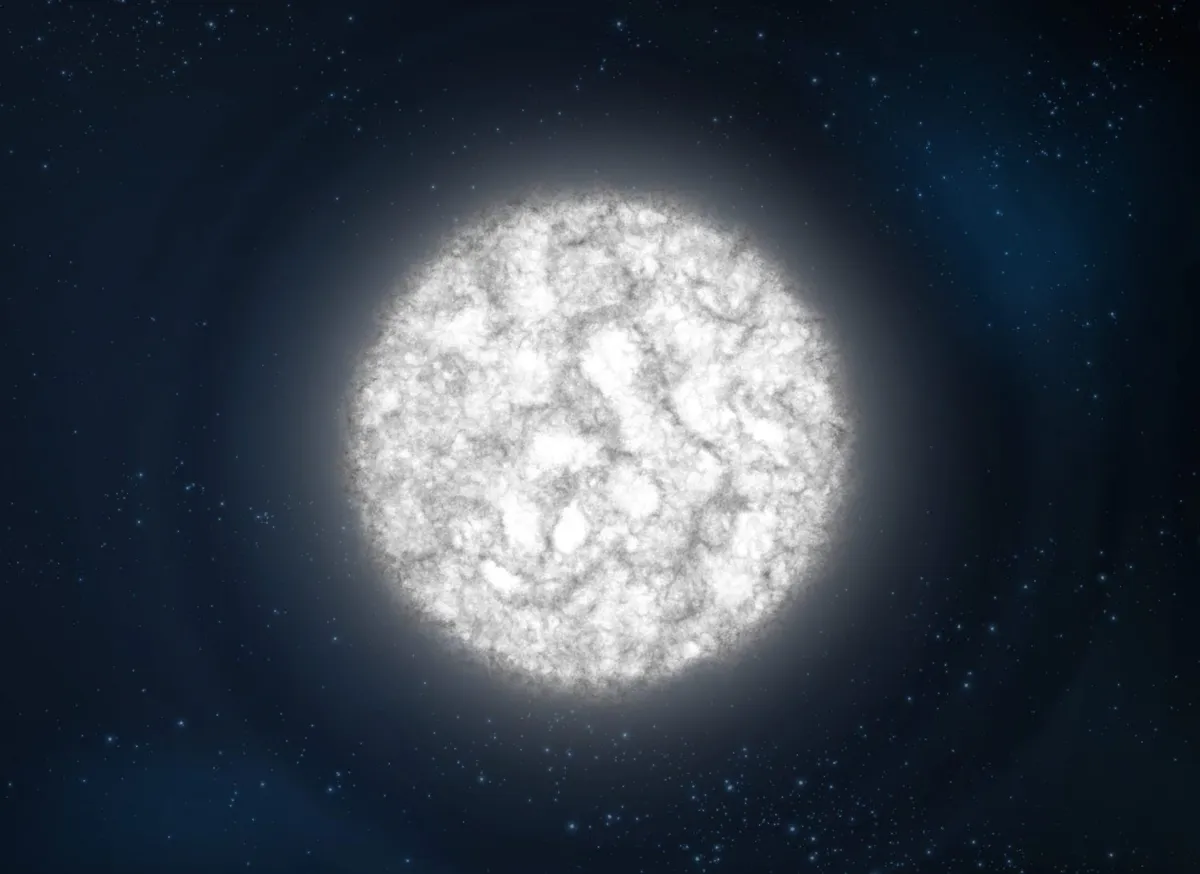
The majority of the carbon and nitrogen in our bodies came from the deaths of small, yellow stars like our Sun.
However, for heavier elements, we must look to even more dramatic stellar events.
While our Sun will die with something of a whimper, larger stars go out with a bang.
The nuclear career of a star more than eight times the mass of the Sun doesn’t end when its core is converted into carbon and oxygen.
Instead, its enormous gravity heats the core to over a billion degrees – hot enough to force carbon nuclei together to make even heavier elements, including neon, magnesium and sodium.
When the carbon runs out – after a piffling 1,000 years – the star undergoes a further series of collapses, heating up and igniting new nuclear fuels: first neon and then oxygen.
On the star’s last day, the core reaches 3 billion degrees, hot enough to trigger its final fusion reaction, the burning of silicon to make iron and nickel.
Iron and nickel are the most stable elements in the periodic table, and so fusing them to make even heavier elements actually consumes energy, rather than releasing it.
This means that once the core has been converted into iron and nickel, the star has twinkled its last.
With no further fusion reactions available to counterbalance gravity, the star implodes, crushing the core into such a small volume that it reaches the same density as an atomic nucleus.
At this point the strong nuclear force fights back against gravity, and the in-falling bulk of the star effectively bounces off the core, creating a shockwave that rips the star apart from the inside and sends a cataclysmic blast of light and matter out into space: a supernova.
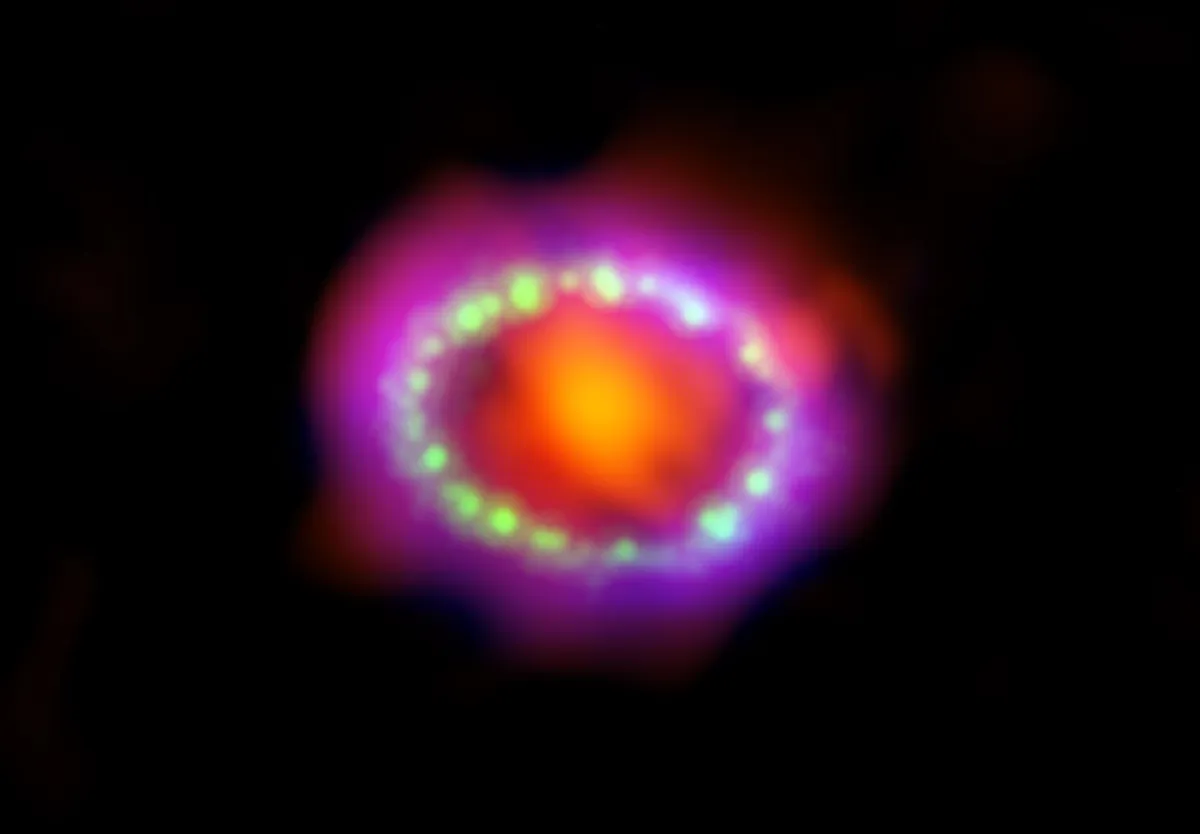
Meanwhile, the core itself collapses into a single, giant atomic nucleus made entirely of neutrons – a neutron star – or, if it is heavy enough, a black hole.
The resulting stellar explosion, known as a Type II supernova, can briefly outshine all the stars in a galaxy.
In the ferocious conditions inside the supernova, further heavy elements beyond iron and nickel are created as lighter nuclei are bombarded by an intense wave of free neutrons, resulting in elements including gallium, germanium, bromine and krypton.
At the same time, particles accelerated to close to the speed of light tear through larger nuclei like bullets, breaking them apart to form much lighter elements like beryllium and boron.
These newly minted nuclei are blasted out into the Universe, along with products of earlier fusion reactions including carbon, oxygen, magnesium and iron, enriching the cosmos with a heady elemental cocktail.
Gravitational waves and gold
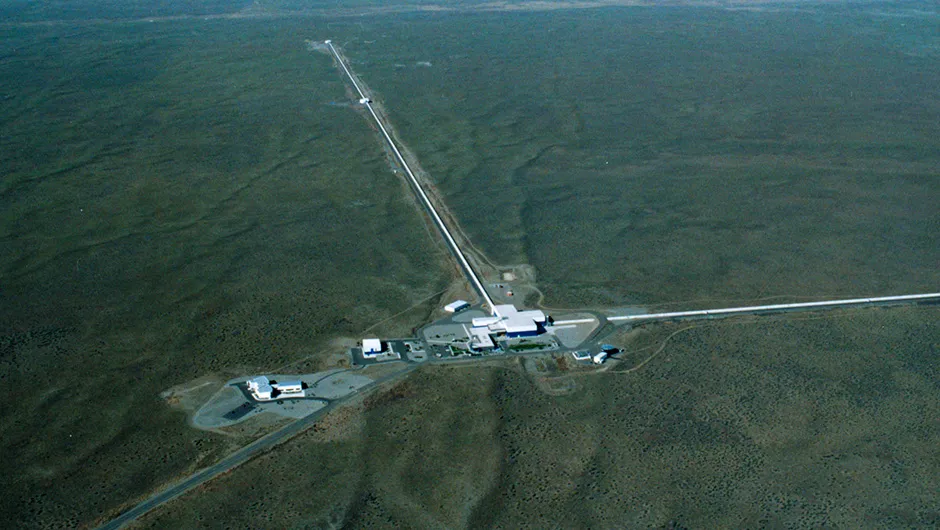
Perhaps the most exciting recent advance in our understanding of the origin of atoms came in 2017, with the discovery by the Laser Interferometer Gravitational-Wave Observatory (LIGO) of gravitational waves released by the collision between two neutron stars.
Along with the gravitational wave, observatories around the world detected light from the awesome collision, revealing large quantities of precious metals, including platinum and gold.
In fact, by one estimate the neutron star collision produced enough gold to make 30 solid-gold planet Earths.
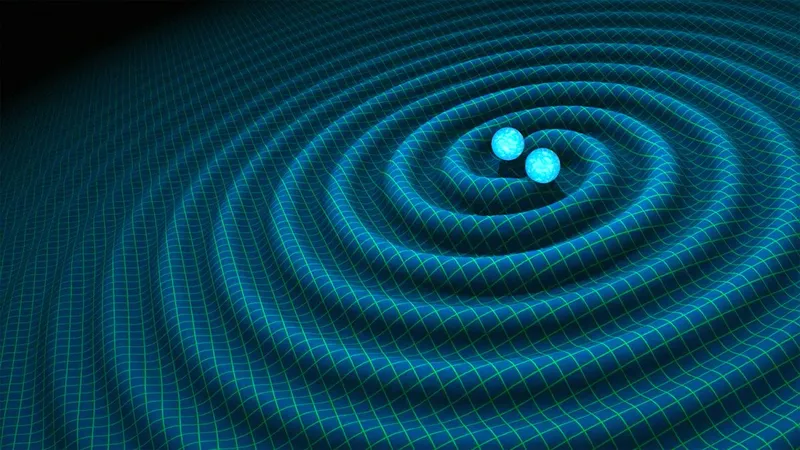
Of course, this gold isn’t floating through space in solid, planet-sized lumps.
Instead the atoms, along with all the other elements created by stars, diffuse out through the nebulous gas that fills our Galaxy.
Over time, knots form in these clouds – stellar nurseries from which clouds of hydrogen, helium and the other elements come together to form new stars.
These are surrounded by swirling protoplanetary discs, filled with all the elements created by previous generations of stars. Over time, these come together to form the planets.
It’s extraordinary to think that everything that made every person, tree, mountain and ocean came from the deaths of stars, billions of years in the past.
This article appeared in the March 2024 issue of BBC Sky at Night Magazine