The early Universe was made of an opaque plasma, a hot sea of ionised gas. It cooled sufficiently for atoms to form about 380,000 years after the Big Bang and the light was free to travel through the Universe almost unimpeded. That light is what we measure today as the cosmic microwave background (CMB).
The Cosmic Microwave Background tells us about the state of the matter it last interacted with all that time ago. It’s essentially a baby picture of the Universe.
Our understanding of the CMB leapt forwards in the 1990s, with the Cosmic Background Explorer satellite discovering tiny fluctuations in an otherwise almost-uniform afterglow, followed by higher-resolution images from balloon-borne experiments.
These density variations have now been mapped over the whole sky, first by NASA’s Wilkinson Microwave Anisotrophy Probe and more recently, at higher resolution, by ESA’s Planck satellite.
Launched in 2009, Planck scanned the sky in nine wavelengths, or colours, of microwave light. It operated for over 4 years and, following its decommissioning in 2013, is now permanently switched off and in orbit around the Sun.

The wealth of data it collected has been pored over by the Planck team and released to the world for other astronomers and cosmologists to study.
It’s allowing insight into what cosmologists call the standard cosmological model, the picture of the composition and evolution of the Universe, starting with a primordial soup of matter and ending with the massive structures we see in the Universe today.
It's thanks to gravity that the tiny temperature and density variations as small as 0.001% in the early Universe – pictured as a seemingly random hodge-podge of hot and cold spots in all-sky maps of the CMB (see at the very top of this article) – expanded and cooled over time to become enormous groups of galaxies arranged in a cosmic web.
Interestingly, there is one place in the Universe that astronomers believe to be colder than the glow left over from the Big Bang, and that's the Boomerang Nebula, known as the coldest place in the Universe.

What is the Universe made of?
In a nutshell, the Universe comprises three main constituents:
- Only around 20% of the matter in the Universe is made of the same stuff we are – atoms, molecules and so on.
- The rest is dark matter, which only feels the force of gravity. But even all this matter only accounts for less than a third of the energy content of the Universe.
- The rest – about 68% – is dark energy, which acts as an anti-gravity force pushing everything apart. However, it has only dominated the Universe in the past few billion years.
One of the most counter-intuitive features of the standard model of cosmology is ‘inflation’: the Universe’s first tiny fraction of a second (about one thousand million billion trillionth), in which it expanded by a factor of around 100 thousand billion trillion.
This smoothed out the visible Universe, making it almost the same everywhere, but it also blew up tiny quantum fluctuations to a macroscopic scale. It’s these fluctuations that led to the density variations in the CMB.
While inflation seems like a theory of convenience, with little or no physical motivation, it does explain many of the observed properties of the Universe.
It’s the best theory we have at the moment, but without firm evidence we could simply be barking up the wrong tree, cosmologically speaking.
While there have been refinements to some of the numbers, the basic model of cosmology hasn’t really changed.
By combining Planck’s precise measurements of the CMB with other large-scale studies of the Universe, cosmologists have narrowed down the half-dozen or so basic parameters to the level of a few per cent, sometimes even less.
For instance, using the latest results from Planck the age of the Universe, calculated as 13.8 billion years, can be determined to an accuracy of less than 0.2%, equivalent to 30 million years.
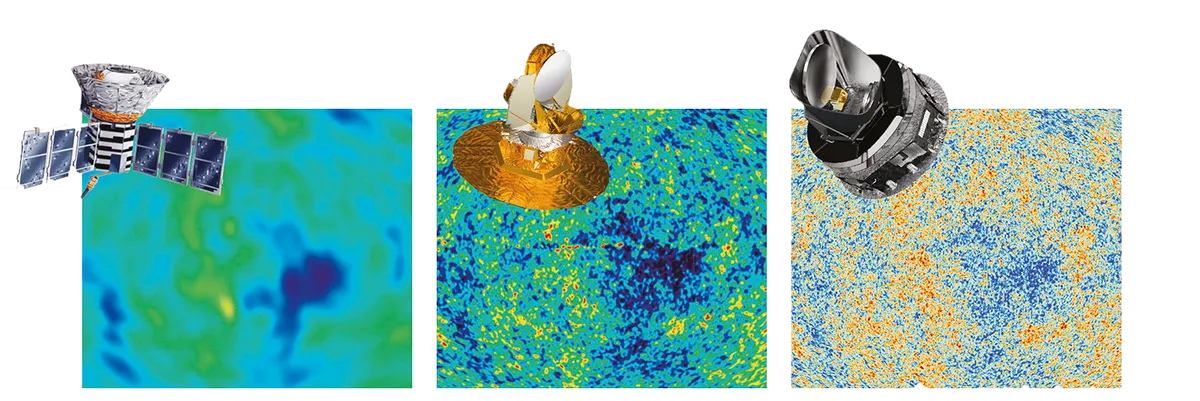
Cosmic interference
Unfortunately, for cosmologists at least, Earth is not completely isolated in the Universe, and the early Universe is not the only source of microwave light.
Material in our own Galaxy, within the Solar System, and even in different galaxies emits light and confuses the picture.
In fact, most of the sky is dominated by emissions from the Milky Way, so separating these ‘foregrounds’ is critical if we are to get a better view of the early Universe.
While the Cosmic Microwave Background looks the same at every wavelength, these other components have a specific range of colours, and so a multicolour view of the Universe can be split up into its constituent parts.
Planck’s most powerful tool is its nine-colour vision, which allows it to separate the cosmic afterglow from the galactic foregrounds with much greater reliability than previous missions, and refine our cosmological parameters more accurately than ever before.
We think of a beam of light as having a wavelength (or colour) and an intensity (or brightness). But in some situations light can also have a preferred orientation, called its polarisation.
What is polarisation?
Light is a wave of oscillating electric and magnetic fields travelling through space. The properties of light we see tell us about the source that emitted it.
In astronomy, for example, the colour, or wavelength, of light tells us about the source’s temperature and the intensity of light tells us the density of the gas or dust that’s emitting it.
If the source has a preferred orientation, then there can also be a preferred direction to the electric and magnetic fields we see, and so a preferred orientation of the light. We call this a polarisation.
The effect can also be created when light is scattered off an object. For example, light reflected of a road is slightly polarised, which is why polarised sunglasses can block out some of the reflected glare.
Light in the early Universe was scattered off electrons and the electric field of the light caused the electrons to oscillate.
If the light had been the same everywhere the electrons would have oscillated in random directions, but the distribution of hot and cold regions in the Universe (which we see as hot and cold spots in the CMB) gave the electrons a preferred direction of oscillation, polarising the scattered light.
The particular structures and patterns in the CMB mean that the observed polarisation has a particular structure – a swirly pattern around hot spots, referred to as an ‘E-mode’.
The distortion of space by gravitational waves would manifest itself as a subtle distortion to the polarisation pattern, adding in a different pattern of swirls, called a ‘B-mode’.
Unfortunately, the signature of gravitational waves is much weaker than the normal CMB polarisation pattern. It’s also masked by other sources, such as gravitational lensing and polarised light from our Galaxy.

What causes light to be polarised?
Light can become polarised if its source has a preferred direction. For example, the galactic magnetic field causes dust particles to be aligned in the same direction, and the light they emit also has this preferred orientation.
Similarly, electrons spiral around the magnetic field lines and emit light with orientations that also correspond to the magnetic field.
While these might be a nuisance to cosmologists, to astrophysicists they’re a fascinating insight into the structure of the Galaxy and the formation of stars.
Light from the early Universe is also slightly polarised, and the pattern of polarisation provides clues as to what happened at the beginning of time, as well as more recently in cosmic history.
There’s little benefit looking at one spot in the CMB, as we don’t know anything about the initial conditions at each point in the Universe.But by averaging them together, and looking at patterns over the sky, we can build up a statistical picture.
The polarisation, or orientation, of the Cosmic Microwave Background has a very particular pattern – it looks swirly. This isn’t that surprising, as the pattern of hot and cold regions on the sky leads to this swirliness.
Multi-coloured Milky Way
Planck’s ability to observe at nine wavelengths makes its data particularly rich. Below are just four of its views of our Galaxy
1
Dust

Interstellar dust grains in our Galaxy glow at submillimetre wavelengths and can be aligned to the magnetic field to give this polarised view.
2
Spinning dust

Dust particles can be set spinning in the galactic magnetic field at a rate of billions of rotations per second, emitting millimetre-wave light.
3
Synchrotron radiation
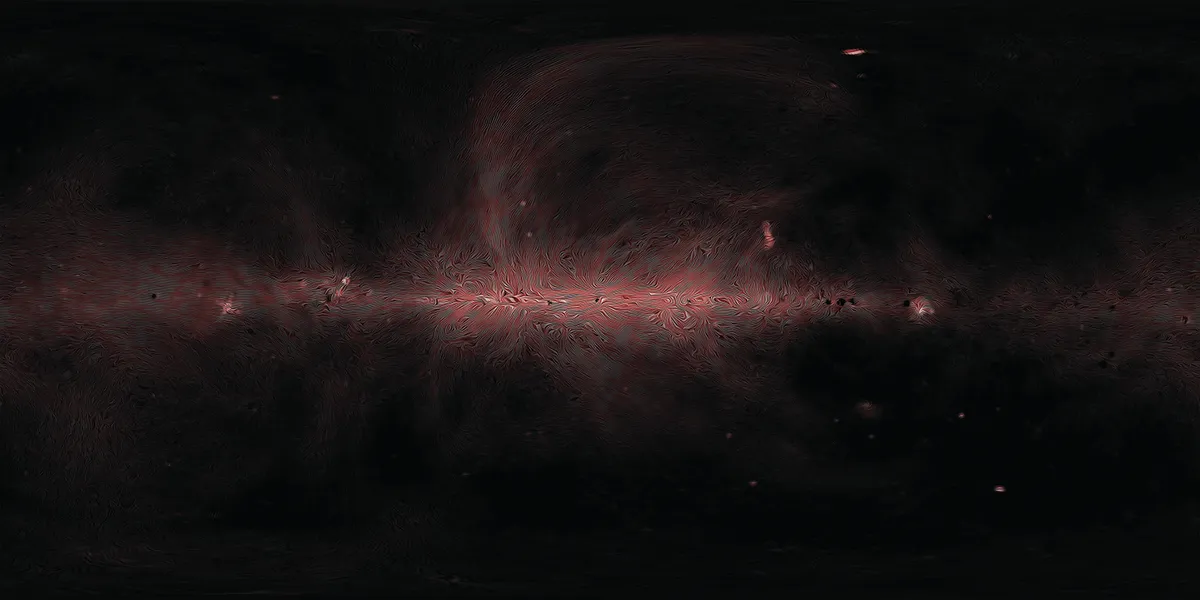
Electrons from within our Galaxy and beyond spiral round the magnetic field lines. As they do, they emit radio waves detected by Planck.
4
Carbon monoxide

The coldest gas in our Galaxy can form molecules such as carbon monoxide. This shows the regions where cold gas is collapsing to form stars.
Looking for patterns in the CMB
Cosmologists are hunting for a much fainter pattern in the orientation, hiding among the swirls.
These so-called ‘B-modes’, if seen in the early Universe, would provide evidence of gravitational waves propagating through the cosmos, originating from the massive expansion of the Universe during inflation.
It is one of the only testable predictions that inflation makes, and finding them would be a huge discovery.
Unfortunately, the expected signal is incredibly weak and is easily masked by a huge number of other effects.
As well as polarised light coming from our Galaxy, the polarisation patterns are also distorted by gravitational lensing, which twists the orientation around and mixes up the patterns.
In March 2014 a team of cosmologists running telescopes at the South Pole reported that they may have found this B-mode signature in their maps of the sky.
The cosmological community, and the rest of astronomy, was buzzing with speculation.
While many were very excited about this detection of gravitational waves, others were more sceptical.
The findings were made by an experiment called Background Imaging of Cosmic Extragalactic Polarization (BICEP2).
It focused on one patch of sky and while it had to compete with the obscuring effects of the Earth’s atmosphere it did so with a large number of detectors – in some ways making it more sensitive than Planck.
Its Achilles’ heel, however, was that BICEP2 only saw one colour of light, and so wasn’t able to confidently separate out the foreground interference.

The assumptions made were too optimistic, and a subsequent collaboration with Planck showed that much of what had been seen was due to dust in our Galaxy, not a signature of inflation.
That’s not the end of the story, though, as the same team are running more telescopes from Antarctica with a greater range of colours.
Combined with Planck’s maps of the galactic emissions, we may yet discover the cosmological B-modes.
There is a huge breadth in the scientific results possible with Planck’s data. Not only from our Galaxy and the early Universe, but also much of the stuff in-between.
When the first stars lit up, their intense light started stripping atoms of their electrons, re-ionising the Universe.
This ionised gas scattered light travelling through the Universe, altering its preferred orientation and subtly distorting our measurements of the CMB.
Planck’s latest results have established that this probably happened about 500 million years after the Big Bang. This is good news for astronomers, as the stars and galaxies that caused the effect should be visible to the James Webb Space Telescope when it is launched.
There is still much to learn from Planck’s observations. While almost all the data from the mission is now available, there is a lot left to understand.
As well as cosmological discoveries, we are just at the beginning of exploring the polarised images of the sky, and astronomers will undoubtedly make many new discoveries about our local neighbourhood.
Dr Chris North is Odgen Science Lecturer and STFC Public Engagement Fellow at Cardiff University. This article originally appeared in the May 2015 issue of BBC Sky at Night Magazine.